The history and science of nuclear energy and the climate problem, part 3: the current state and potential of nuclear energy
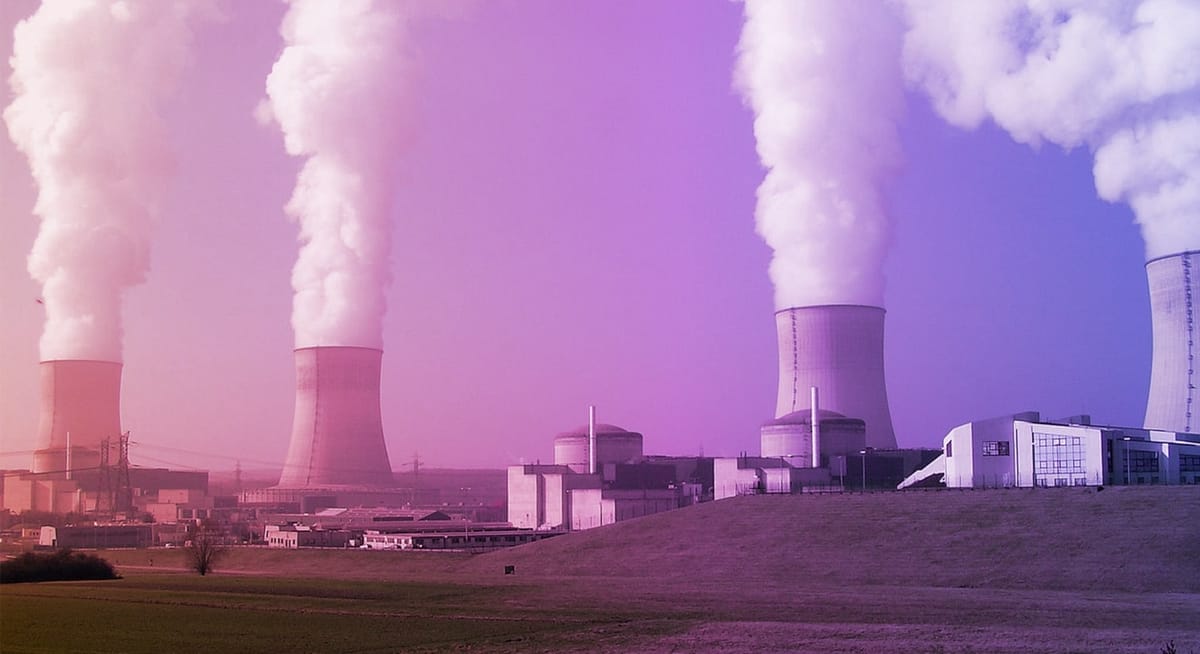
Q: Would most other nations, including Russia, have done the same thing we did, confronted with the same opportunity to use the bomb?
A: Look, answering this question would be pure speculation. I can say this, however: By and large, governments are guided by considerations of expediency rather than by moral considerations. And this, I think, is a universal law of how governments act.
Prior to the war I had the illusion that up to a point the American Government was different. This illusion was gone after Hiroshima.
Perhaps you remember that in 1939 President Roosevelt warned the belligerents against using bombs against the inhabited cities, and this I thought was perfectly fitting and natural.
Then, during the war, without any explanation, we began to use incendiary bombs against the cities of Japan. This was disturbing to me and it was disturbing many of my friends.
Q: Was that the end of the illusion?
A: Yes, this was the end of the illusion. But, you see, there was still a difference between using incendiary bombs and using the new force of nature for purposes of destruction. There was still a further step taken here - atomic energy was something new.
I thought it would be very bad to set a precedent for using atomic energy for purposes of destruction. And I think that having done so we have greatly affected the postwar history.
Szilard makes an interesting point here about governments, which would a fascinating topic for a different article, but let me say this much about it here; I sure hope he is wrong about this “universal law”, I would hope that a hypothetical future workers government in a war with a fascist nation would make a different choice, and not resort to such drastic measures as wiping whole cities of the face of the earth.
Asides from that, Szilard’s prediction about the precedent that was set by the atomic bombings and the nuclear arms race are spot on. To this day, one cannot talk about nuclear energy without someone bringing up nuclear weapons, the two are intrinsically connected in the minds of many people. However, to what extent is this link really fundamental from the point of view of physics? We will explore this question further in this third part.
Let’s start with the working principle behind nuclear energy, it is in fact relatively simple. One takes a relatively heavy atom, shoots a neutron into it causing it to become unstable and split into two (or more) parts. This process releases energy because the resulting products combined have a lower energy than the original starting atom (this is true for all product elements heavier than Iron). The energy is released in the form of heat, some neutrons, photons (gamma radiation), electrons (beta radiation) and Helium ions (alpha radiation). The released neutrons will collide with other atoms, causing those to split as well, and thus even more heat to be produced. And so a nuclear chain reaction is created. The resulting massive amounts of heat can be used to drive a turbine and generate electricity.
A couple of things are important. First of all, for every neutron adsorbed at least one neutron should be released again, otherwise the chain reaction will die out. On the other hand, too many neutrons will cause the amount of reactions to increase exponentially, which becomes a problem when the amount of heat generated becomes a lot bigger than the amount of heat that can be removed. If the reactor’s fuel is in a solid state, this can eventually cause the fuel to melt, a meltdown!
Not only the amount of neutrons, but also the speed of these neutrons is important. Some fuels are more susceptible to collide with slow neutrons, others are more stable (i.o.w. have a larger energy barrier) and only spit upon collision with faster neutrons. To control this speed so called neutron moderators are used, which slow down the neutrons, ideally without absorbing too many of them.
Therefore, the art of controlling a nuclear reactor, is the art of controlling the amount and speed of the neutrons in the reactor. Some reactors are designed such that physics will cause the amount of neutrons to automatically decrease when the fuel gets too hot, such reactors are called inherently safe.
Thorium, Uranium and Plutonium
This brings us to a first design choice: the fuel. As a general rule of thumb, all odd isotopes of the actinides are directly usable as a fuel in a nuclear fission reactor. The actinides are the elements in the bottom row of the periodic table of elements, and are the heaviest elements. An isotope is a version of an element with a different number of neutrons in the nucleus. As an example, Actinium, the first actinide, consists of 89 protons, and either has 136, 137 or 138 neutrons. These isotopes would then be called Actinium-225, Actinium-226, and Actinium-227, where the number behind the element name is the sum of the protons and neutrons (89+136=225, 89+137=226 and 89+138=227).

Figure 6: Periodic table of elements, encircled are the elements relevant for nuclear reactors: wikimedia commons.
Of these actinides only two can be found on earth in relevant amounts: Thorium and Uranium. Uranium is found in a mix consisting mostly of 99.27% Uranium-238 and 0.72% Uranium-235, the slightly lighter Thorium on the other hand consists of 99.98% Thorium-232 and is 4 times as common on earth. Of these three isotopes only Uranium-235 has an odd number of neutrons and is directly able to sustain a nuclear chain reaction by itself, we therefore call it fissile. The other two mentioned isotopes, Thorium-232 and Uranium-238, are not directly fissile. However, they are fertile, meaning that they can be converted into a fissile isotope by capturing neutrons (Uranium-233 and Plutonium-239 respectively, both formed after capturing one neutron and emitting two electrons, i.o.w. double beta decay).
Some reactors or other applications require a larger percentage of Uranium-235 in the fuel than can be found in natural Uranium ore. Mostly these are designs where too many neutrons are absorbed by things that are not fissionable, such as the moderator or cooling fluid. In such cases it is necessary to increase the amount of neutrons in the reactor to ensure that every fission will still cause at least one other fission. One way to do this is to increase the concentration of the fissile part of the fuel, or in other words, in the case of a Uranium fuel, to increase the concentration of Uranium-235 compared to Uranium-238, this is what is called enrichment. How much enrichment is required depends on the application. For bombs you’ll want as much explosive power in as little as mass as possible, which means that you’ll want the concentration of Uranium-235 to be as large as possible, in other words you’ll want weapons-grade Uranium (more than 85% Uranium-235). On the other hand, if the aim is to create Plutonium you’ll want the bare minimum of enrichment, since it is the Uranium-238 that will produce the Plutonium-239 upon neutron capture. For electricity production only low amounts of enrichment are required (less than 20%), and some electricity reactor designs don’t need any enrichment of the fuel at all and can use natural Uranium (CANDU and other pressurized heavy-water reactors), such reactors have other ways to ensure that there are enough neutrons in the reactor (such as the use of heavy water as neutron moderator).

Figure 7: Decay reactions for creating Uranium-233 and Plutonium-239 from Thorium-232 and Uranium-238 respectively.
That being said, Uranium-235 is not the only possible fuel. Thorium-232 , was already shortly mentioned above, unlike Uranium-235, it will not fission immediately upon capturing a neutron, instead it will absorb this neutron and become Thorium-233. This Thorium-233 is very unstable and will very quickly decay to Protactinium-233 by emitting an electron (beta radiation). The Protactinium-233 will then decay in the same way again to form Uranium-233, which is fissile and will therefore split and release energy upon capturing another neutron. Note the difference between decay and fission, the two are often confused. Radioactive decay is the general term for any unstable isotope releasing any kind of radiation to become more stable. Fission is a type of radioactive decay, which sometimes occurs spontaneously in heavy unstable isotopes, but can also be induced by neutron capture as we have seen above. So all fission is radioactive decay, but not all radioactive decay is fission, other forms of radioactive decay are; the emission of a highly energetic photon (gamma radiation), the emission (or capture) of an electron (beta radiation), or the emission of a Helium-4 ion (alpha radiation). The fission products that are created by the splitting of an unstable isotope, are of course significantly lighter but are often still unstable isotopes and therefore will often still undergo some form of (alpha, beta, gamma) radioactive decay before eventually becoming a stable isotope.
A similar process to what we have seen for Thorium-232 occurs in Uranium-238, which becomes Uranium-239 after capturing a neutron. This decays to Neptunium-239, and again to Plutonium-239, which is fissile. Uranium fuelled nuclear reactors will always contain some Uranium-238 (how much depends on the amount of enrichment), and therefore such reactors will always produce some Plutonium-239 as a byproduct. This is not a problem because Plutonium-239 is also fissile and is therefore a fuel for the nuclear reaction, and as such can just be ‘burned up’ as well. Plutonium, however, has another more sinister application, which is where it gets its bad reputation from.
Plutonium-239: The isotope of choice to kill and destroy
All three of the active fuel isotopes mentioned above are in principle also usable to create a nuclear weapon; Uranium-235, Uranium-233 (from Thorium-232) and Plutonium-239 (from Uranium-238). However, in practice the element of choice for this application is almost always Plutonium-239. This is because the minimal mass (critical mass) required to sustain a chain reaction in Plutonium-239 is 5 times smaller than that of Uranium-235. This means that a Plutonium based weapon can be significantly lighter than a Uranium based one. Additionally, it is a lot easier to separate Plutonium from Uranium than it is to enrich natural Uranium to the high concentrations of Uranium-235 required for weapons-grade Uranium.
The third isotope, Uranium-233, is comparable to Plutonium-239 in terms of critical mass, but requires a purity that is 1300 times higher to make a functional weapon. This is because the nuclear reaction that produces Uranium-233 also produces a bit of Uranium-232 as a byproduct. This Uranium-232 produces highly energetic gamma radiation which interferes with electronics and therefore significantly limits the applicability of Uranium-233 in weapons. A similar problem occurs in the production of Plutonium-239, where there will always be a bit Plutonium-240 produced as a byproduct. This Plutonium-240 is problematic as well because it tends to spontaneously fission, producing neutrons which could make the bomb go off prematurely if the concentration of Plutonium-240 is too high. However, the amount of Plutonium-240 that is acceptable in a Plutonium-239 based weapon is 1300 times higher than the amount of Uranium-232 in a hypothetical Uranium-233 weapon.
So if you’d want to make nuclear weapons you’d choose Plutonium-239 as the active isotope, and if you can’t get your hands on that, you’d revert to highly enriched Uranium. It is thus no coincidence that the first large scale nuclear reactors that were built both in the US and the USSR were Plutonium production reactors. These reactors never produced any electricity, they couldn’t because they were not designed to do this and therefore lacked the necessary infrastructure to convert heat to electricity. Furthermore, to some extent the goals of creating Plutonium-239 or electricity are opposites. Because to create Plutonium-239 the fuel should not stay too long in the reactor, otherwise the Plutonium-239 would be ‘burned up’ or too much Plutonium-240 would be created. If such a reactor would be optimized for the production of electricity instead, the fuel would be replaced way less often, because in this case it would in fact be beneficial for the Plutonium-239 and Plutonium-240 to fission as well, and therefore create additional heat and electricity. In fact, the longer you can keep the fuel in the reactor the more of the heavy actinides produced will fission again, which means more heat and thus electricity produced, and also that less of these heavy actinides will end up as nuclear waste (the heavier actinides are usually problematic in the waste because of their long life times).
After the first Plutonium production reactor opened in 1943 (Hanford, US) it took 11 years before the first electricity producing reactor became operational (1954, Obninsk, USSR). The difference in timespan with how long it took to build the first Plutonium production reactors, once again shows us how skewed the priorities were. Furthermore, almost all of the early reactors that did produce electricity, only had this as a secondary goal, where the primary aim was to create Plutonium. Historically speaking nuclear energy is clearly a byproduct of nuclear weapons, however it would be a mistake to assume that this implies that this link is fundamental and also true from the viewpoint of physics. The political and historical context caused certain choices to be made and priorities to be set, but choices and priorities they definitely were and nothing more than that. It would in fact have been possible to completely develop nuclear energy without ever making a single bomb.
When one aims to make a bomb, one optimizes the reactor and the surrounding infrastructure (e.g. enrichment and other separation facilities) for this purpose and tries to create as much Plutonium-239 as possible, and of course this has had its effect on which designs and ideas received the most funding and were eventually implemented, the consequences of which can still be seen today. In the next section we will explore one of the many alternate paths not taken: Thorium.
Thor’s Hammer: an unused power that never got its due attention
Weinberg realized that you could use thorium in an entirely new kind of reactor, one that would have zero risk of meltdown. ... his team built a working reactor .... and he spent the rest of his 18-year tenure trying to make thorium the heart of the nation’s atomic power effort. He failed. Uranium reactors had already been established, and Hyman Rickover, de facto head of the US nuclear program, wanted the plutonium from uranium-powered nuclear plants to make bombs. Increasingly shunted aside, Weinberg was finally forced out in 1973.
Science writer Richard Martin writes this about physicist Alvin Weinberg. Weinberg filed the patent on a conceptual light water reactor together with Eugene Wigner, to this day the most common type of nuclear reactor. Light water, as opposed to heavy water, is just regular water and is used in this type of reactor as a neutron moderator and as a coolant. The use of light water requires that the Uranium fuel is slightly enriched, since light water absorbs more neutrons than heavy water.
Weinberg questioned the wisdom of the continued upscaling of his own design, because he understood all too well the critical weakness in his design. If something were to go wrong, such as a critical failure in the water pumps, and the cooling would become inadequate, then the fuel rods could overheat and melt: A Meltdown! For the original application this design was made for, the navy, this wasn’t really a problem because it is easy to guarantee the availability of water for a boat or submarine, since it is quite literally swimming in its own cooling fluid. However, Weinberg was not a big fan of applying his design on land. Expressing his concerns cost him his job as director of the Oak Ridge National Laboratory, Weinberg himself writes:
[Congressman] Chet Holifield [head of the Joint Committee on Atomic Energy (JCAE), which oversees the US Atomic Energy Commission (AEC)] was clearly exasperated with me, and he finally blurted out, "Alvin, if you are concerned about the safety of reactors, then I think it may be time for you to leave nuclear energy." I was speechless. But it was apparent to me that my style, my attitude, and my perception of the future were no longer in tune with the powers within the AEC.
For applications on land Weinberg proposed a different kind of reactor, one which did not use any water, one using Thorium as the fuel instead of Uranium, one that was inherently safe. This design was based on earlier designs made for the air force, and though a nuclear propelled aircraft never became a reality the design itself was tested successfully on land. The test reactor at the Oak Ridge National Laboratory used Uranium-233 (made from Thorium) dissolved in a Fluoride salt as the fuel, and operated successfully from 1965 to 1969, and in doing so, demonstrated the feasibility of the concept.
Such a liquid Fluoride Thorium reactor has several advantages. First of all, the lack of water automatically removes the risk of contaminated water from the reactor entering the ecosystem. Secondly, and most importantly, there is 0 risk of a meltdown. For one because the fuel is already in a dissolved liquid state in normal operation. Furthermore, such a reactor includes a plug made from that same Fluoride salt which will melt when the Fluoride-fuel solution in the reactor gets too hot. The melting of this plug will automatically drain the reactor and store the solution safely in large container tanks. No human or electronic action is required, just gravity. Once the solution is outside of the reactor it can expand, which will automatically cause it to cool down. To start the reactor up again, all one has to do is pump the solution back into the reactor.
Additionally, such a reactor produces almost no Plutonium, or other heavy actinides for that matter. Because for this to happen Uranium-233 would have to capture 6 consecutive neutrons and become Uranium-239 so it can decay to Plutonium-239. The odds that an Uranium-233 atom does this are very low, since for every neutron capture there is a chance that the isotope will fission instead. And the capture-to-fission ratio of Uranium-233 is smaller than that of Uranium-235 and Plutonium-239. Moreover, due to its liquid nature, the heavy actinides that it does produce can just stay behind in the reactor and be burned up, only the fission products have to be extracted as waste.
Furthermore, the radioactivity of the waste that a liquid Fluoride Thorium reactor produces returns to background levels way faster than that of a conventional light water reactor. Because, as we saw earlier, it produces less heavy actinides, which are the most problematic isotopes in nuclear waste. 83% of the waste from a liquid Fluoride Thorium reactor has a half-life of a few hours to days, the remaining 17% only has to be stored for 300 years compared to the ten thousands of years required for the waste from more conventional reactors. Compared to the Uranium fuel cycle, the Thorium fuel cycle has a radiotoxicity that is 10.000 times smaller.
Moreover, the liquid Fluoride Thorium reactor operates at low pressures, and the pressure does not increase a lot even if the reactor runs several hundreds of degrees too hot. Thorium is four times as abundant as Uranium. And the liquid nature of the reactor allows for refuelling and separation of the waste fission products without having to turn the reactor off.
Sadly, Thorium never truly got its chance to shine. The technology for Uranium reactors was already established, and Thorium could not satisfy the military’s demand for Plutonium. Thus, in 1973, the US pulled the plug on the research into Thorium.
Nuclear fusion energy: the energy source of the future?
Nuclear fission energy is popularly abbreviated to nuclear energy. However, nuclear energy is wider than just nuclear fission energy, it encompasses all energy generation involving nuclear reactions, and as such also includes nuclear fusion energy. No article on nuclear physics could be complete without at least talking a bit about nuclear fusion, so let’s dive into that here.

Figure 8: Binding energy as a function of the size of the nuclei.
As we have seen, very heavy nuclei release energy when they split, the opposite is true for the very light nuclei that gain energy when they fuse together. The middle between these two extrema is at Iron-56, which has the highest binding energy, indicating that of all the nuclei this is the most stable one and would take the most energy to completely take apart. Figure 8 shows this binding energy as a function of the size of the nuclei.
It is exactly such a nuclear fusion process that takes place in the sun (and other stars). Four Hydrogen-1 nuclei fuse together to eventually form one Helium-4 nucleus. The process releases radiation and an enormous amount of heat which keeps us warm and provides us with light on earth. In theory this process could be replicated on earth to create heat and thus electricity. However, the technical challenge is the enormous pressure and the temperature of millions of degrees Celsius required to accomplish this.
Once again an explosive history
Some authors condemn nuclear fission energy because of nuclear weapons and waste, only to praise nuclear fusion energy in the next paragraph as the alternative that is free of all those things that make nuclear fission nasty. This is unbelievably and painfully ironic, first of all because nuclear fission actually does produce some radioactive waste. And secondly because many nuclear weapons actually are based on nuclear fusion, the so called thermonuclear weapons or hydrogen bombs.
The fusion reaction produces quite a lot of radiation, and this radiation is absorbed by the structure of the reactor itself, upon absorbing this radiation some of the nuclei in the structure will become different nuclei which may be unstable and radioactive. The end result is that, first of all the structural integrity of the reactor degrades over time, and secondly that at the end of the lifetime of the reactor some of the structure will remain radioactive for about 100 years. Granted, this is a relatively short time period, but the claim that nuclear fusion produces no radioactive nuclear waste is plain false.
The history of nuclear fusion starts in 1933, when Mark Oliphant was able to create Helium-3 using a proton-accelerator. And as if the corruption of nuclear fission was not enough this breakthrough would soon also lead to new even more destructible weapons. The first Soviet atomic bomb, in 1949, came way sooner than the Americans expected, and so the Americans quickly started designing a new type of bomb that would top the existing fission based bombs. The idea was simple, take an existing Uranium-235 bomb and add some Deuterium and Tritium (Hydrogen-2 and Hydrogen-3 respectively). After the first stage, the Uranium stage, would explode, the temperatures would quickly become high enough for the Deuterium and Tritium to fuse, releasing more energy and more neutrons, the extra released neutrons would in turn cause even more fission in the Uranium, releasing even more energy.

Figure 9: Fireball of the Tsar Bomba.
On the 25th of May 1951, a first proof-of-concept bomb successfully exploded on an island in the middle of the pacific ocean. It proved to be twice as destructive, compared to the same bomb without the fusion phase. Shortly afterwards, on the 1st of November 1952 the first true thermonuclear bomb exploded, which was 450 times more destructive than the bomb that was dropped 7 years earlier on Nagasaki. However, this explosion pales in comparison to the bomb that the USSR brought to explode over Nova Zembla on the 30th of October 1961. The code named Tsar bomb was 5 times as powerful as the before mentioned American thermonuclear bomb, and resulted in a fireball of more than 8 kilometres wide. The resulting mushroom cloud was 67 kilometres high, 7 times higher than Mount Everest. The heat from the explosion was still noticeable at distances of 270 kilometres, windows shattered as far as Finland and Norway. To this day, this was the most powerful man-made explosion, an eyewitness describes:
The clouds beneath the aircraft and in the distance were lit up by the powerful flash. The sea of light spread under the hatch and even clouds began to glow and became transparent. At that moment, our aircraft emerged from between two cloud layers and down below in the gap a huge bright orange ball was emerging. The ball was powerful and arrogant like Jupiter. Slowly and silently it crept upwards... Having broken through the thick layer of clouds it kept growing. It seemed to suck the whole Earth into it. The spectacle was fantastic, unreal, supernatural.
The future?
Just as with nuclear fission, the military research into nuclear fusion has spawned a parallel research into applications for the production of electricity. And, just as with nuclear fission, there are a lot of different reactor designs both with their own advantages and disadvantages, a full comparative analysis of which would be outside of the scope of this article. The interested reader can find more information in the many links dotted throughout this article.
What all designs have in common is that a huge amount of energy is required to get the fusion reaction to start. And none of the reactors which have been built so far have come close to breaking even. The world record was set by the Joint European Torus near Oxford, this reactor operated for about one second in 1997, consumed 700 megawatts of electricity, to produce 16 megawatts of heat. Despite that this was not even near breaking even it was still a major accomplishment. And in 2007 the research was continued by the start of the International Thermonuclear Experimental Reactor project in southern France. The expectation is that when this reactor is finished, it will be able to operate for about 1 second in 2035, consuming 300 megawatts of electricity to produce 500 megawatts of heat.
Perhaps in the far future nuclear fusion will be able to contribute to the electricity generation, however we cannot expect this to happen any time soon. Nuclear fusion is still very much in the experimental phase, and with the promise of only 1 second of operation in 2035, we cannot expect nuclear fusion to be able to contribute to the energy transition at all. Additionally, it is very difficult to find funding for such long-term projects under capitalism where short-term profits are the norm, and as such promises such as X megawatts of electricity in 20YZ should always be taken with a grain of salt because such promises are made in the context of trying to lure in funding within a system that expects short-term results. Personally, I would be very surprised if nuclear fusion would be able to contribute to the electricity generation in any significant amount within my lifetime.
Do you value this free article? Please consider a onetime donation or subsribe to read all of my content. Thank you!